Media
Transcript
I think I’ve said this before, but today it is worth repeating: one of the most amazing things about the universe is that we have the capacity to understand it. More or less, it seems to behave in predictable ways that are governed by the basic forces and that are communicated through set particle interactions. Our current particle and field theory explanations are less than 150 years old, and in that time we have gone from just starting to understand electricity to living in a technologically advanced world that has to take relativity into account as we travel from place to place using the GPS receivers in our phones.
While I feel comfortable with our big picture understanding of the evolution of our universe, I have to acknowledge that there are places where our understanding is, at best, hand-wavy and vague. One of those places is the period of time between the release of the cosmic microwave background radiation and the establishment of the large scale structure of galaxies and galaxy clusters.
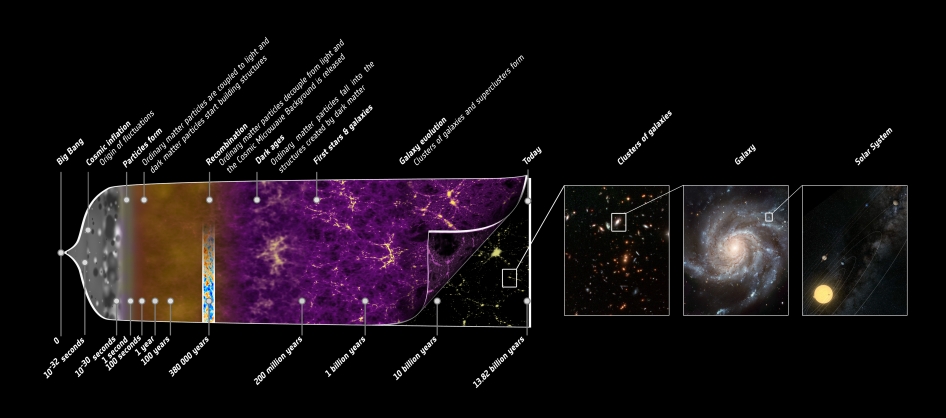
As the story goes, our universe emerged hot and tiny, but over time expanded, and as it expanded, it cooled. What started as pure energy settled into a soup of constantly colliding electrons, photons, and atomic nuclei. This was the situation for several hundred years, and because light couldn’t travel any significant distance without being absorbed during a collision, the universe during these early eras was opaque.
About 400,000 years after the Big Bang, however, the universe cooled enough that electrons and nuclei could come together to form neutral atoms, and at that moment, it became possible for photons to fly away unhindered. This happened everywhere in the universe, and today, we see photons from that moment as the Cosmic Microwave Background (CMB). It is the stage after the CMB formation that we are struggling to understand.
Initially, there was a cosmic dark age, during which nothing was giving off light, and the primary activity of the universe was the gravitational collapse of slight areas of higher density material. The gas that made up the universe was neutral, and the light of the first stars had to ionize that gas to make the universe into the largely transparent reality we have today. What we are struggling to understand is how fast, and how did the universe go from almost uniform gas to being highly structured.
Numerous theories and computer simulations have worked to simulate the collapse of neutral gas clouds and the formation of galaxies and their supermassive central black holes. Some of the best theories, in my opinion, depict this as a turbulent process, where some of the largest galaxies in the early universe were able to form before the universe was a billion years old. This matches our observations of galaxies that appear fully formed when the universe was only 800 million years old.
It’s good when reality matches theory. The problem is, the more we observe, the more we have been finding galaxies at ever earlier epochs, and things have started to go from matching our expectations to challenging us to make the universe form structures faster than we thought possible.
In December 2017, scientists led by Eduardo Banados announced the discovery of a quasar that was fully formed just 690 million years after the Big Bang. With an 800 million solar mass central black hole, it is a massive system for its time, but it is still small enough that its formation is explainable. This remarkable system also allowed scientists to begin to measure what fraction of the universe was reionized. It was a beginning, and it was a stretch, but all was well with our understanding of the universe.
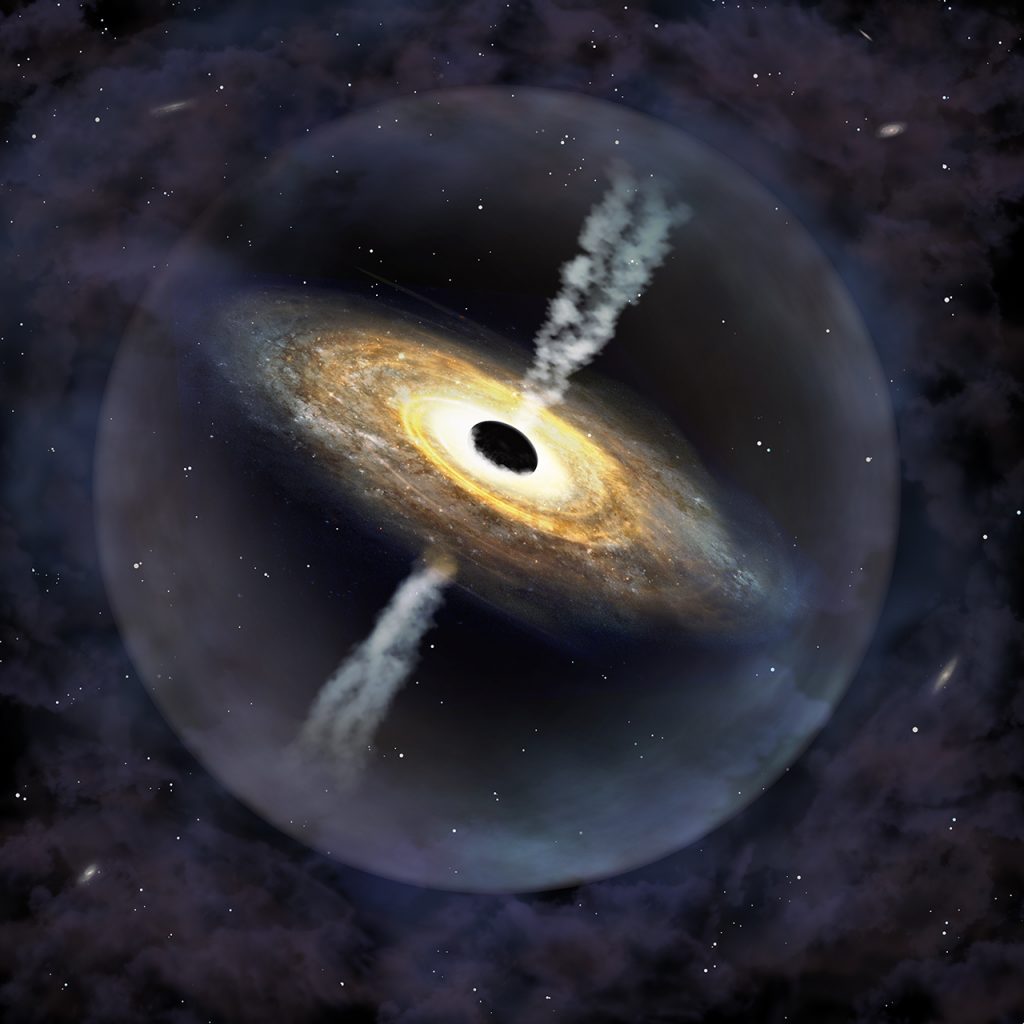
That is a whole lot of background information that allows me to now talk about today’s first news story. In a new paper accepted to Astrophysical Journal Letters, a team with first author Jinyi Yang has announced the discovery of another super distant quasar, this one seen 700 million years after the Big Bang. It is the second farthest and is remarkably larger. Its central supermassive black hole is 1.5 billion solar masses, making it twice the size of the 2017 discovery. For something to be this big this early in the history of the universe, the team believes that a 10,000 solar mass black hole needed to have formed and been available to seed the quasar’s growth just 100 million years after the Big Bang.
This is a much larger black hole then we had been thinking could already exist at that point, and it would have needed to form through the singular collapse of a massive cloud of gas. How something this big forms that fast is going to present new challenges: heat released during gas collapse should throttle down the collapse, all else being equal, so there must be mechanisms that enable the collapse somehow, or perhaps they formed in a wholly different way.
While this discovery raises a lot of questions about supermassive black hole formation, it also provides some answers on the age of reionization. According to the paper on this discovery, the neutral gas fraction was about 40% at this quasar’s time, and the quasar’s host galaxy was forming a remarkable 210 solar masses worth of stars per year. This is giving us an understanding of the patchy nature of the universe’s reionization and is showing us just how frenetic early star formation actually was.
Studying this second-most-distant quasar was non-trivial and required using all the most massive telescopes on Mauna Kea in Hawaii, including the Keck, Gemini, and UKIRT telescopes. As part of new efforts to better bring together Hawaii’s indigenous peoples, their culture, and modern astronomy, a group of thirty teachers got to name the object. They came up with the name Poniua‘ena, which means “unseen spinning source of creation, surrounded with brilliance” in the Hawaiian language. These were Hawaiian language immersion school teachers taking part in the Imiloa Astronomy Center of Hawaii’s A Hua He Inoa program.
These kinds of surprising details in how our universe was able to rapidly form objects inform how we have to look at structure formation and are reminders that while we have a big picture understanding of our universe, there are a lot of gaps in our understanding, and while we have given some of the gaps cool names like dark matter, they are nonetheless massive missing pieces of the puzzle.
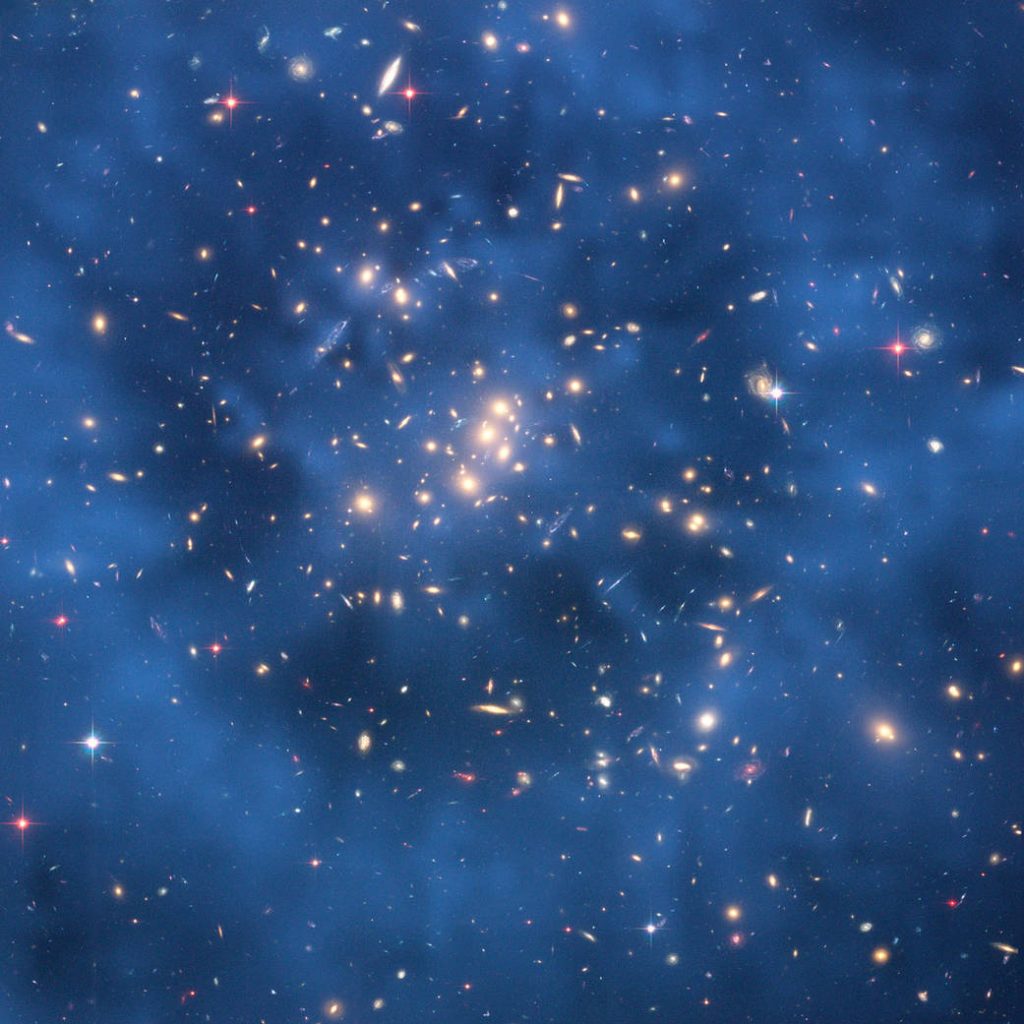
Since the 1930s, astronomers have known that there is invisible stuff out there that is gravitationally affecting its surroundings while neither absorbing nor emitting light. For the past many, many years, scientists have been looking for weakly interacting massive particles (WIMPs) that could explain this missing mass. So far nothing has been found, and many, many theories have been ruled out as these massive particles fail to show up in underground vats or high energy particle collisions.
One theory that is slowly starting to gain traction is the possibility that the theoretical particle the axion might be that missing dark matter particle. Axions were first theorized in the 1970s as the particles that are behind a field that causes neutrons to always be electrically neutral and otherwise helps explain why we don’t see particles violating the charge-parity relationship. Axions, if they have a small amount of mass, could also be a constituent of dark matter – a very, very low-mass constituent.
In large enough numbers, even the lowest of mass particles could be dark matter. The issue has been that theories couldn’t find a way to generate enough axions to generate dark matter. Until now.
Here, I’m going to read from the press release put out by the Institute for Advanced Study at Princeton because I can’t explain this better than they already have:
By assigning a nonzero initial velocity to the axion field, the team discovered a mechanism—termed kinetic misalignment—producing far more axions in the early universe than conventional mechanisms. The motion, generated by breaking of the axion shift symmetry, significantly modifies the conventional computation of the axion dark matter abundance. Additionally, these dynamics allow axion dark matter to react more strongly with ordinary matter, exceeding the prediction of the conventional misalignment mechanism.
Two members of the research team, Keisuke Harigaya and Raymond Co, previously explored the concept of axion dynamics in the study “Axiogenesis,” which explained how the excess of matter over antimatter could be due to a nonzero initial velocity of the QCD [quantum chromodynamics] axion field. This study also provided a framework for generating new insights into the questions surrounding dark matter.
What I really like about this theory is that axions were already needed for reasons that have nothing to do with dark matter, and there have been some observations that hint at seeing the effects of axions, but the quality of the data isn’t enough to say they exist with certainty. I will take a particle that has multiple reasons to exist and low signal / not entirely believable observations over a custom-needed particle with no observations any day. This is starting to remind me of the early days of looking for the Higgs boson where teams were seeing hints of it in data, but the noise was such that they couldn’t say anything with certainty. This is the first time in a long time I’ve felt hope that we might be able to identify dark matter.
We still have a long way to go. We need definitive observations, and the theories need more work, but this feels like the start of something, and we will be following this story and bringing you new results as we have them.
Learn More
Monster Black Hole Found In The Early Universe
- The University of Arizona press release
- NOIRLab press release
- W.M. Keck Observatory press release
- The Current news article (UC Santa Barbara)
- “Poniua‘ena: A Luminous z = 7.5 Quasar Hosting a 1.5 Billion Solar Mass Black Hole,” Jinyi Yang et al., 2020, Astrophysical Journal Letters (Preprint on arxiv.org)
Case for Axion Origin of Dark Matter Gains Traction
- The Institute for Advanced Study press release
- “Axion Kinetic Misalignment Mechanism,” Raymond T. Co, Lawrence J. Hall & Keisuke Harigaya, 2020 June 26, Physical Review Letters
Credits
Written by Pamela Gay
Hosted by Pamela Gay
Audio and Video Editing by Ally Pelphrey
Content Editing by Beth Johnson
Intro and Outro music by Kevin MacLeod, https://incompetech.com/music/